When it was first discovered in 2012, the Higgs boson captured the popular imagination and became the subject of intense scientific scrutiny. One way scientists knew for sure that the object they discovered was indeed the long-theorized Higgs particle involved identifying one of its unique qualities: It is the only “scalar” fundamental particle researchers have ever seen. Unlike every other elementary particle we know of—every electron, quark and neutrino—the Higgs boson has a quantum spin of precisely zero.
Gleaning the full significance of this unique characteristic requires that we think bigger than the boson itself, an object so hopelessly unstable it decays into less massive and less exotic particles almost as soon as it blinks into existence. Instead, we must turn our attention to the realm of quantum field theory, and to the special place the Higgs boson calls home, the Higgs field. Along the way, we’ll see how the relationship between quantum fields and the basic physical property of quantum spin can help us better understand the nature of our universe. And why finding the Higgs is far from the end of the story.
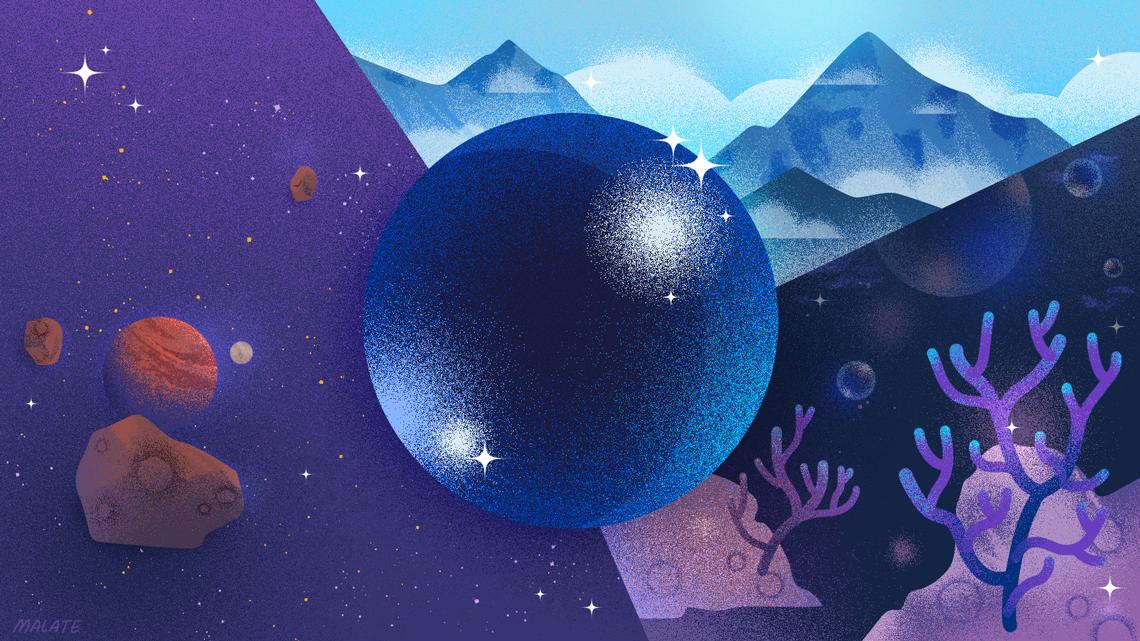
Taking a spin in quantum field theory
We’ve established that the Higgs boson is a spin-zero, a.k.a. “scalar” particle, but what does that mean? And what does that have to do with the Higgs field?
Let’s start with the basics: Quantum field theory posits that all particles, spinning or not, are local excitations or fluctuations within “quantum fields.” These fields play an important role in enforcing the laws of nature.
“From the quantum field theory perspective, when we are thinking about everything, we think that everything is made up of fields,” says Nausheen Shah, associate professor of physics at Wayne State University. “That's the electromagnetic field, gravitational field—but also all the particles, the electron field, the Higgs field—all of these are fields that are permeating space.”
There’s a quantum field for each of the 12 known matter particles, as well as fields for all four of the fundamental forces (corresponding to force-carrying particles like the photon).
In most cases, we need two numbers to describe a field at any given point in space and time. One number indicates its strength or “magnitude;” the other indicates its “spin.” Spin here is a quantum property that doesn’t have an easy analogue at the macroscopic scale. Particles don’t spin the way the Earth spins, but particles and planets do share something in common—they both have angular momentum.
“And that momentum is finite. You can measure it,” says Matt Strassler, a physicist at Harvard University.
Even though a particle isn’t actually rotating around an axis, it moves and interacts with other particles and forces as if it were. The Higgs boson, which has no spin, does not. But what about the Higgs field? How can a field have a spin?
“A field having spin, that is really a question of whether the field ‘points’ or not,” Strassler says.
A gravitational field, for example, points toward the massive object that is generating it. So the gravitational field that holds you to the Earth points—from your perspective—down, toward the center of the planet.
The Higgs field, on the other hand, is just as spinless as the Higgs boson. Like a college senior sitting forlorn in a career counselor’s office, it has no direction.
It’s no coincidence that both the Higgs boson and the Higgs field are spin-zero. “The properties of a particle are essentially properties of the field,” says Peter Onyisi, associate professor of physics for the University of Texas at Austin. Like waves in the ocean, “Higgs bosons are vibrations in a Higgs field.”
Tipping the scalar: the Higgs field & rotational symmetry
The fact that the Higgs field has zero spin is an important element of the Higgs mechanism—the way the Higgs field gives particles mass. “The source of mass for fundamental particles, it has to be spin-zero,” Shah says. “Physics has to be the same if you translate, or rotate, or boost to a different frame [of reference]. It just has to be.”
To understand what Shah means by this, we must examine another special trait of the Higgs boson—its nonzero vacuum expectation value. A field’s vacuum expectation value is the average value of all the information you can have about that field, including its magnitude and spin, across a macroscopic region of space.
If you traveled deep into some intergalactic void and tried to measure the vacuum expectation value of the gravitational or electromagnetic field thousands of lightyears from the nearest galaxy or star, you would likely find it to be negligible. That’s because most quantum fields have a vacuum expectation value of zero unless a particle is present.
The one exception to this rule is the Higgs field. The Higgs field has a nonzero vacuum expectation value throughout all of spacetime, meaning there is always some value associated with it, even when no Higgs particles are present. What’s more, that value is constant, meaning you can measure it at any place or time, and you’ll always get the same answer.
Things would get pretty weird if the Higgs field had a spin, because not only would it have a nonzero vacuum expectation value everywhere at once, but it would also point in a uniform direction that could be measured from anywhere in the universe. This would mean that we could define a preferred direction, a “true north,” for all of spacetime.
Such an idea goes against some of our most basic precepts about the universe itself, including the idea of “isotropy” or “rotational symmetry,” which tells us that the laws of physics remain uniform no matter which direction we face. This isn’t just a theory; it’s an observational fact. Scientists have spent decades searching to no avail for violations of rotational symmetry.
“You’re literally saying that if you’re in a frame, and you did a rotation, whatever physics you’re seeing here are not the same when you’re looking at [a different angle],” Shah says. “It just doesn’t make any sense.”
But where did the spin go?
For our understanding of physics to work, the Higgs cannot have a spin. But it’s still strange for a fundamental particle to be spin-zero. So, theorists have come up with ways that it might not be.
For example, it could be that the Higgs is not actually a fundamental particle at all. Some models speculate that the Higgs boson is a composite particle, made up of two or more non-scalar fundamental particles whose spins add up to zero.
“The Higgs being a composite particle has a very long history as an idea,” says Onyisi.
He says composite Higgs models appeal to theorists because they address another peculiarity of the Higgs—its incomprehensible mass at high energies.
Physicists want to understand how particles behave at high energy scales, in part because those energy scales mimic the conditions precipitated by the Big Bang—and thus can give us a view into what our universe was like at its very beginning.
A particle can have very different properties, depending on the energy scale at which it is measured—differences in charge, mass and other quantum numbers. When it comes to changes in mass, some particles are “protected” in the mathematical theory of particle physics. For example, the photon has no mass, and it does not suddenly gain a mass as energy scales increase.
The Higgs boson, however, does not enjoy such protection. “Famously, there’s a runaway behavior at high energy scales of the Higgs mass term,” Onyisi says.
Calculations predict that a fundamental Higgs particle would be impossibly massive at such an energy scale.
A composite Higgs could present an easy solution to this problem, since the fundamental particles making up a composite Higgs would presumably have their mass controlled via some well understood physical mechanism. “So that actually is, for a lot of people, a very attractive idea,” he says.
What if the Higgs really is fundamental, though? Could it be that it does have a spin after all, one that is hidden from us in an extra dimension? Some models say so.
Strassler says alternative theories of the Higgs all share a common problem: It’s hard to get them to line up with what we already know about the particle and its field. “What makes the Higgs field and the Higgs boson really tough is that we also have to arrange that it has some other rather weird properties,” Strassler says.
There’s a big difference between the mass of a top quark, the heaviest fundamental particle discovered so far, and the mass of an electron, one of the lightest. To get their differing masses, particles have to interact to different degrees with the Higgs field—and any new theory about the Higgs has to be compatible with that observable reality.
“It is quite difficult,” Strassler says, “to make theories that cook up the Higgs boson as a [particle that spins in a 4th dimension], or cook it up as a composite object, and still successfully get it to have all the other properties that it needs to have in order to give the particles of the Standard Model the wild diversity of masses that they have.”
Scalar fields forever
If alternative models are wrong, it could be that the Higgs is indeed the lone fundamental particle with no spin.
Or it might not be alone after all. “Even in the world of the Higgs boson itself, it’s not clear that there’s only one of them,” Onyisi says.
Some models of grand unified theories—which suggest that three of the four fundamental forces (all except gravity) may have been joined together as one unified force during the high energy period following the Big Bang—predict the existence of a whole family tree of Higgs bosons.
“The Higgs field solves a certain class of problem,” Onyisi says, “and so often what people do when they encounter that kind of problem theoretically is [they] just replicate the idea for the [new] context.”
It’s also possible the early universe was once teeming with a different kind of scalar boson, but those particles have since disappeared.
That’s how scientists view the inflaton, a particle associated with cosmic inflation. “So the idea is, right after the Big Bang, there was this very short, like 10-30 seconds, period of what’s called inflation,” Shah says.
This is the period during which the very fabric of spacetime itself exploded out from the starting point of the Big Bang, inflating at a pace faster than the speed of light to form the hot, dense soup of the early universe. What powered that explosive growth could have been something called the inflaton field, with associated particles called inflatons.
Of course, the inflaton and the inflaton field would need to be spin-zero, “because there can’t be a direction which is associated with any point in space,” Shah says.
The inflaton field also would have had a nonzero vacuum expectation value, at least during the inflationary period.
Some scientists think inflation ended when the inflaton field began to lose its vacuum expectation value, a process that would have produced an abundance of inflaton particles. These particles would have quickly decayed into the ordinary matter that makes up the universe today.
Shah says this could explain the incredible homogeneity we observe in the night sky. “When we look out to the universe all around us, as far as we can see, everything is very, very homogenous,” Shah says. “And [without inflation,] there is zero reason for that. In fact, it doesn’t work with our current understanding of things.”
That’s because if we look far in two opposite directions in space, we’re looking at segments of the universe that, without inflation, would have never, ever been in causal contact. In other words, they would have never been close enough to interact, so there’s no reason they should look alike.
But if the inflaton field existed everywhere at once in the early universe, and if it lost its vacuum expectation value at a uniform rate throughout all of space, it would make sense that things should look relatively similar in all directions.
The investigation continues
As we’ve seen, the Higgs and its lack of spin answer some questions about our universe, but they also raise new ones. Shah says she keeps studying the Higgs sector in the hopes of answering this one: In a world made up of only a handful of subatomic ingredients, why do so many exotic, unstable particles exist?
“The Standard Model of particle physics is something we have built empirically over the past 100-ish years,” she says. “And if you actually look at what the world around us is made of, it is actually just one tiny subset of these particles”—just electrons, neutrinos and two types of quarks. This is the first “generation” of known matter particles.
However, more particles exist. Each of the particles that make up our atoms have relatives, making up two additional particle generations.
The next two generations each contain copies of the particles we see in the first, but those copies are much more massive, in some cases several orders of magnitude. Scientists can produce and study these particles in accelerators like the Large Hadron Collider, but they are too massive to remain stable and disintegrate into less massive particles almost immediately after they are born.
“Why in the world should how they interact with this background field [the Higgs field] be so different? Where does it come from?” Shah says. “I feel like—and this is personal, obviously—that there are secrets…We’re not seeing the full picture.”